All published articles of this journal are available on ScienceDirect.
Color Spectrographic Analysis of Respiratory Sounds: A Promising Technology for Respiratory Monitoring
Abstract
Background:
The need for reliable respiratory monitoring has increased in recent years with the frequent use of opioids for perioperative pain management as well as a high prevalence of patients suffering from respiratory comorbidities.
Objective:
Motivated by the success of acoustical color spectrographic techniques in other knowledge domains, we sought to build proof-of-concept systems for the computer-based color spectrographic analysis of respiratory sounds, recorded from various sites.
Methods:
We used a USB miniature electret microphone and a Windows-based color spectrographic analysis package to obtain color spectrograms for breath sound recordings from the neck, from an oxygen mask, from the ear canal, and from a leak-free microphone pneumatically connected to the cuff of a laryngeal mask airway.
Results:
Potentially useful color spectrographic displays were obtained from all four recording sites, although the spectrograms obtained varied in their characteristics. It was also found that obtaining high-quality color spectrograms requires attention to a number of technical details.
Conclusion:
Color spectrographic analysis of respiratory sounds is a promising future technology for respiratory monitoring.
1. INTRODUCTION
The need for reliable respiratory monitoring has existed since antiquity, but this need has become especially pressing in recent years with the frequent use of opioids for perioperative pain management and an increased prevalence of patients suffering from respiratory comorbidities, such as sleep apnea [1-5].
Given this crucial need, efforts at developing a simple, dependable method of continuous respiratory monitoring have been ongoing for a great many decades. Early efforts were based on direct visual observation of chest and abdominal movements as well as observing signs, such as nasal flaring, or the use of the accessory muscles of respiration. Later, with the invention of stethoscope, simple acoustical methods of respiratory assessment became available to the clinical community. With the subsequent invention of electronic amplification, a variety of new technologies became available, including respiratory monitoring belts placed on the chest and abdomen [6-9], nasal pressure methods [10, 11], nasal / oral thermistor methods [12, 13] (the thermistor warms up with expired gases), nasal / oral capnography [14, 15], extraction of respiratory information from the photoplethysmograph signal [16, 17], and electrical impedance methods based on a small injected electrical current [18, 19].
Out of these respiratory monitoring methods, capnography is amongst the most common and clinically used for continuous respiratory monitoring but suffers from a need to continually ensure that the gas sampling system is operating correctly [20]. Extraction of respiratory information from the pulse oximeter photoplethysmograph signal remains a field of active research [21] but has not yet come into the mainstream. A device known as ExSpiron 1Xi operates by detecting electrical impedance changes in the thorax and respiratory muscles and seems to be particularly promising [22], but is neither simple nor inexpensive.
One possible technology for continuous respiratory monitoring, that has interested the author for some time, is the use of color spectrographic analysis of respiratory sounds. Motivated by the success of color spectrographic techniques in the diagnostic monitoring of rotating machinery [23] and the analysis of bird calls [24], the author set about to build his own system for the color spectrographic analysis of respiratory sounds.
2. MATERIALS AND METHODS
2.1. Technique
Much of the earlier work at our laboratory utilized a battery-operated RadioShack 33-3013 miniature omnidirectional electret microphone Fig. (1) secured to a stethoscope head (or another device) via a short piece of tubing (Fig. 2) and then amplified using an ordinary audio amplifier, such as a RadioShack miniature audio amplifier (Catalog # 2771008). Following amplification, the signal is then fed to the “line input” of the host computer for further signal processing.
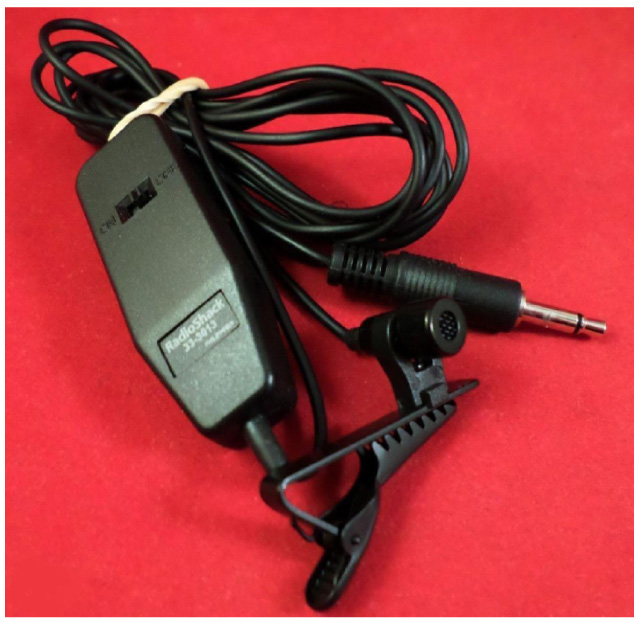
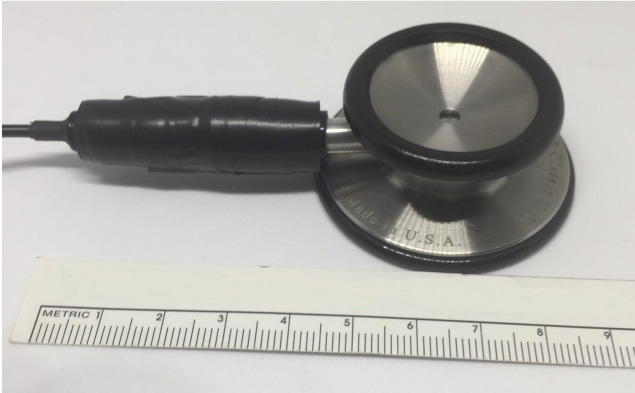
While this arrangement works well, the recent commercial availability of USB-connected miniature electret microphones (Fig. 3) has made it even easier to obtain quality bio-acoustical recordings. This is because USB microphones require no built-in battery (it obtains its power instead from the USB connection) and contains an internal amplifier as well. An additional advantage of USB type microphones is that the final signal obtained does not depend on the characteristics of the analog and digital circuitry within the host computer. One potential disadvantage, however, is the lack of a gain adjustment control in the built-in amplifier in most USB type microphones.
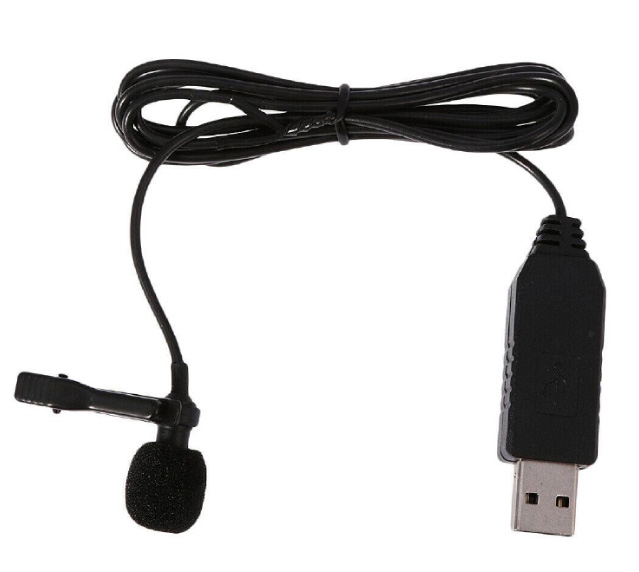
For time-domain digital signal processing software, we have been using a free software program known as Audacity, an open-source digital audio editor and recording application package available for the Windows, macOS/OS X, and Linux operating systems. The program can be downloaded at https://www.audacityteam.org. Three special features of this program are likely to interest bio-acoustics investigators [1]: an Amplify feature that scales the signal [2], a Normalize feature that “normalizes” the signal to a chosen maximum amplitude (e.g., 0 dB), and [3] three digital filtering options (Low Pass Filter, High-Pass Filter and Notch Filter). However, many other excellent free audio software packages are also available - for a comprehensive list visit https:// en.wikipedia.org/ wiki/ Comparison_of_free_software_for_audio.
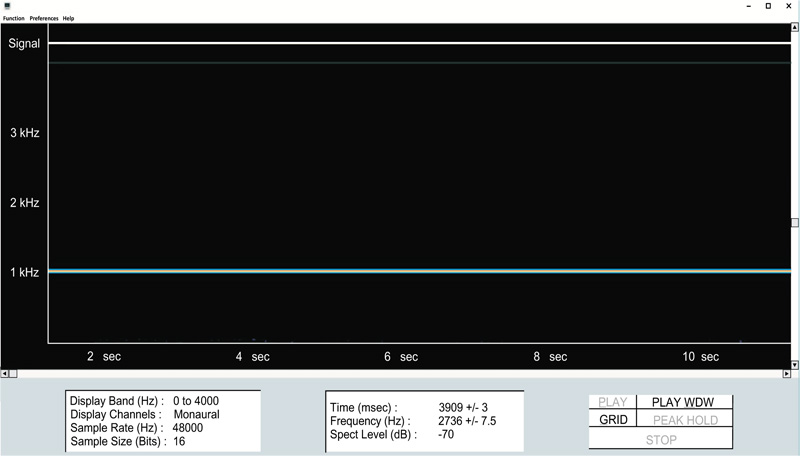
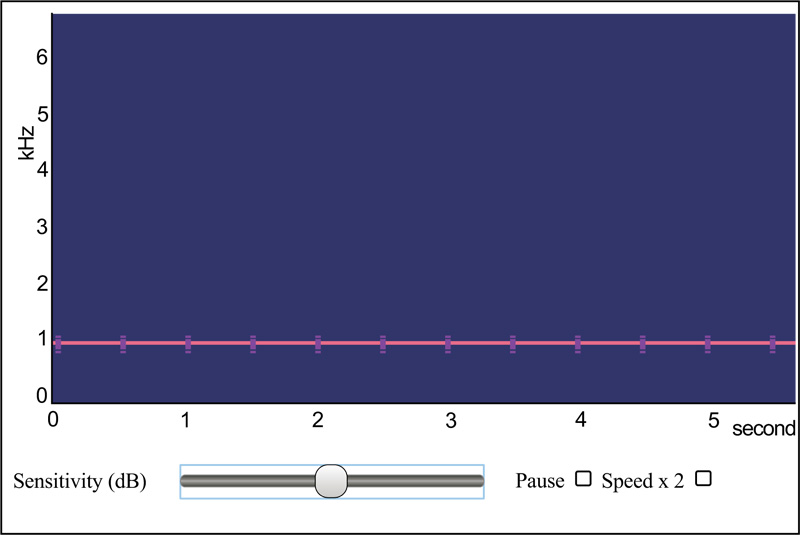
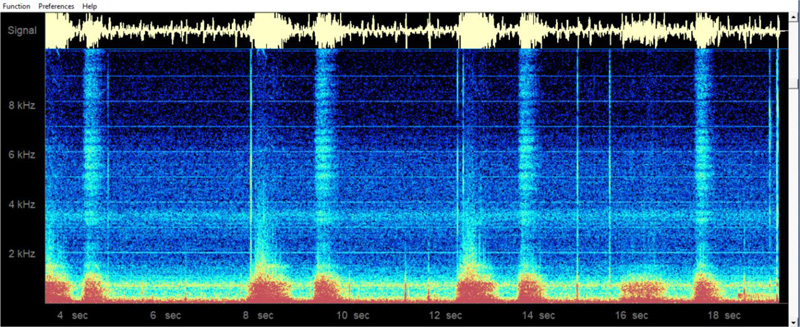
For color spectrogram digital signal processing software, we have primarily been using a Windows program called Spectrgram16 (Fig. 4), available as a free download at https://auditoryneuroscience.com/sites/default/files/gram16_setup.zip.
Another color spectrogram program (a Web-based JavaScript app) that may interest researchers is available at https://auditoryneuroscience.com/acoustics/spectrogram (Fig. 5). This program has the advantage that it does not require to be downloaded and installed, but suffers from the disadvantage that it does not work with all Web browsers.
With this background, let us consider a series of projects that were initiated to explore the potential value of displaying color spectrograms for breath sound recordings.
3. PROJECTS
The first project sought to obtain color spectrograms for breath sound recordings from the neck. This recording location offers an important advantage over recording from commonly used sites, like the anterior chest wall, in that respiratory sounds recordings from the neck are generally not contaminated by heart sounds.
Fig. (6) shows a sample neck recording in the time-domain as well as the corresponding frequency-domain color spectrogram. The Masimo Acoustic Respiration Rate (RRa) monitor similarly provides a non-invasive continuous monitoring of respiration rate via a neck acoustic transducer [25-28], although their proprietary system does not offer an analog acoustic signal for user scrutiny or for computer analysis, and nor does it offer any form of color spectrogram analysis. Additionally, the potential value of recording breath sounds from the neck can be seen from reports of tracheal sound recordings being helpful in detecting apnea in patients recovering from anesthesia [29], as well as useful in other clinical settings [30, 31].
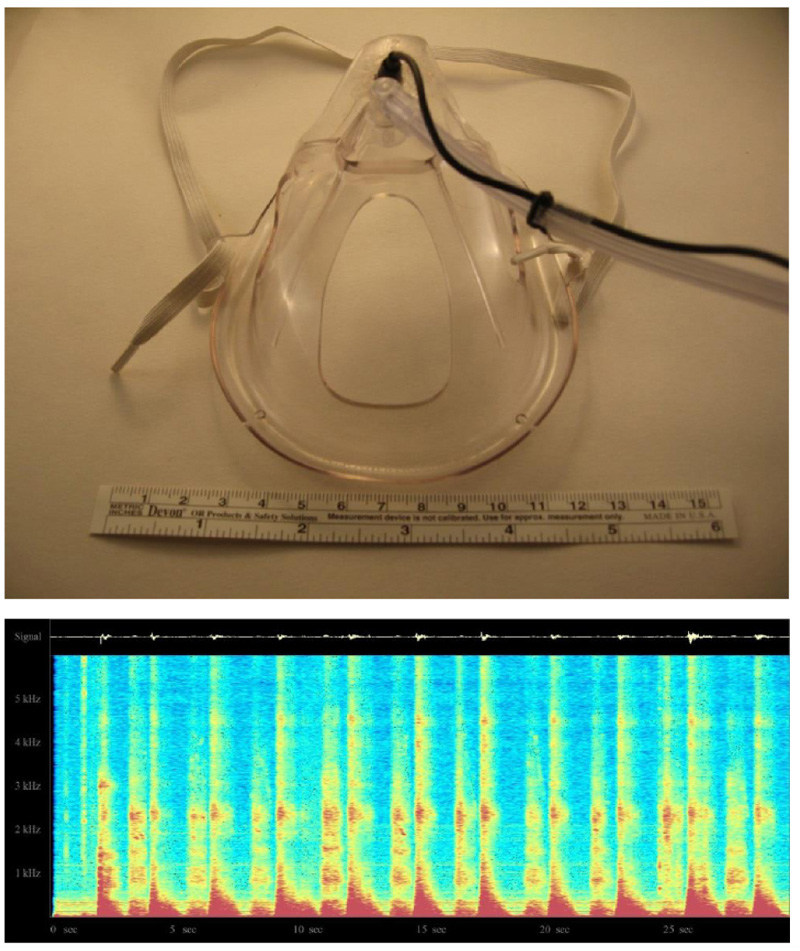
The second project sought to embed a microphone into an oxygen mask to record respiratory sounds (Fig. 7, top). As before, the amplified signal was fed into the color spectrogram program, spectrogram16.exe, to produce a display such as that shown in Fig. (7). Again, note that both inspiration and expiration are clearly visible.
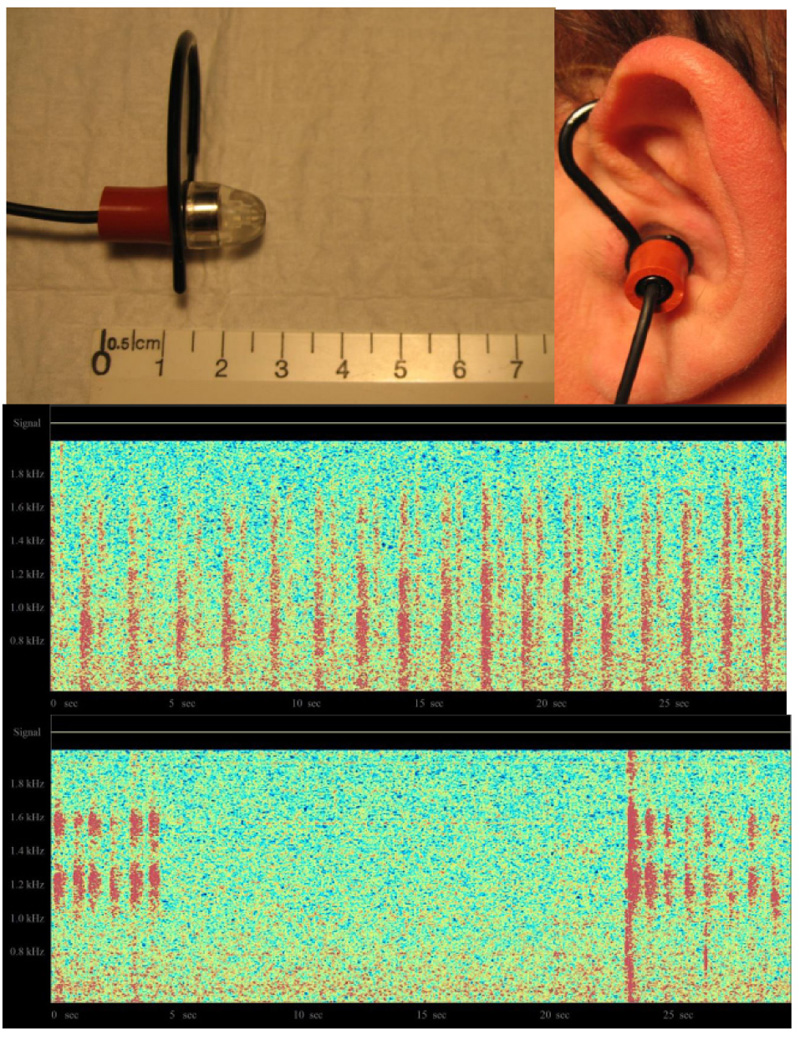
The third project sought to record respiratory sounds from the ear canal. In this case, a miniature electret microphone was modified with the addition of an adapter to allow it to be placed comfortably in the external ear canal. The amplified signal was once again connected to a real-time color spectrogram program. The top of Fig. (8) shows the modified microphone assembly, while the bottom shows the obtained respiratory acoustic spectrograms, under two different conditions.
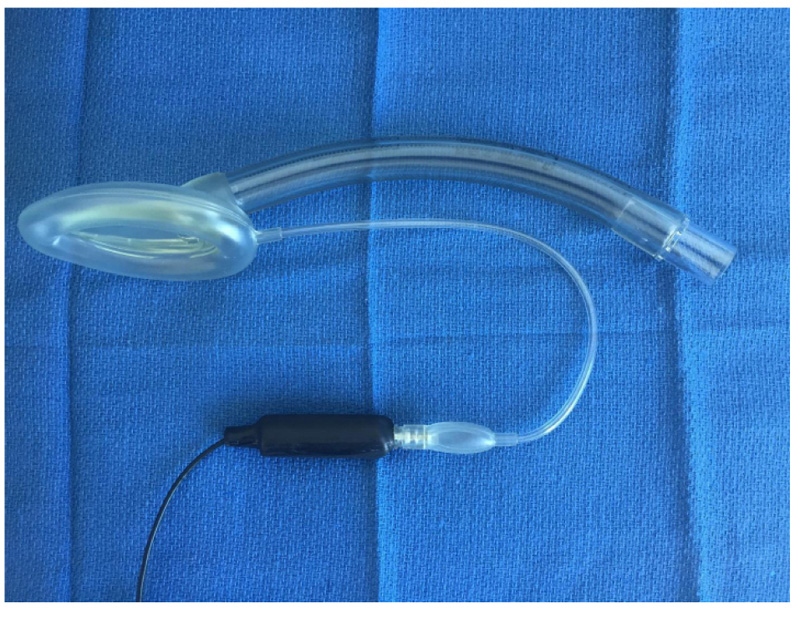
The final project was to add a special microphone to a Laryngeal Mask Airway (LMA) using a custom-built leak-free microphone assembly based on a miniature electric microphone (Radio Shack 30-3013, Radio Shack, Fort Worth, TX). This microphone was embedded in a shortened 3-ml plastic syringe with a Luer lock end and epoxy glue was used to hermetically secure the microphone into the barrel of the syringe (Fig. 9). A high-gain monaural audio amplifier (Radio Shack Mini Audio Amplifier/ Speaker 2771008) was then used to amplify the microphone signal for use with a headset or for computer analysis [32].
In an earlier study [32], the system was evaluated by the author in 10 patients under general anesthesia, using the classic LMA, with patients undergoing spontaneous ventilation. In these cases, clearly identifiable regular breath sounds were obtained, although in one patient who developed partial airway obstruction with the LMA in situ, the sounds become chaotic, irregular, and intense. A sample normal audio recording may be downloaded at http://lmamonitor.homestead.com. Fig. (10) shows the color spectrogram display from this recording obtained using the free Windows spectrogram program spectrogram16.exe.
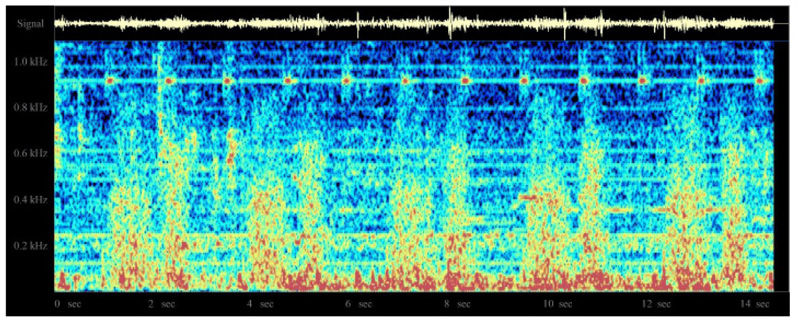
4. RESULTS AND DISCUSSION
A color spectrogram is a plot of the frequency components of a signal against time, with the signal intensity encoded as a color. In the images shown, red areas indicate strongest signal levels, while blue areas are the weakest non-zero signal points: BLACK < BLUE < GREEN < YELLOW < RED.
Several ideas for recording breath sounds were explored in this paper, with the focus on exploring various locations on the body as acoustic recording sites for use in color spectrographic analysis. The expected value of these efforts is that with experience, clinicians will be able to interpret the obtained raw sounds and corresponding color spectrograms to assist in the detection of various normal and pathologic states. These include normal breathing, tachypnea, phonation, partial airway obstruction, wheezing, and ventilation leaks with positive pressure ventilation. It is further envisioned that the real-time display of color spectrogram breathing patterns, locally or at a central monitoring station, may eventually turn out to be a useful means of remote respiratory monitoring in patients at an increased risk of respiratory depression.
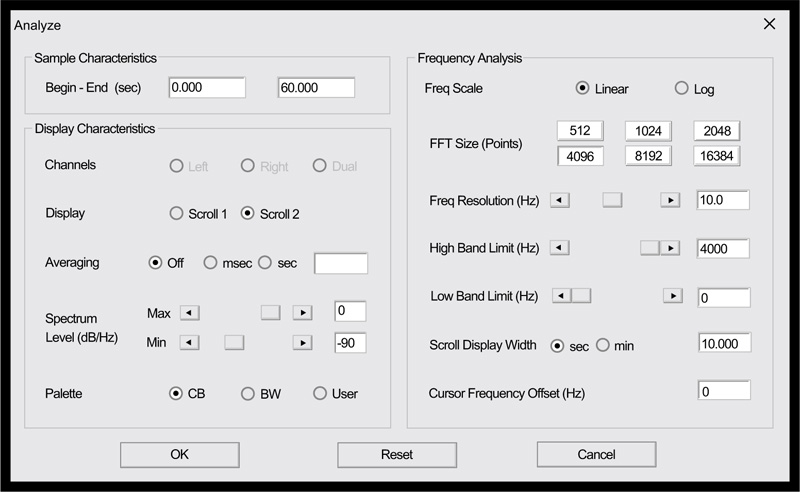
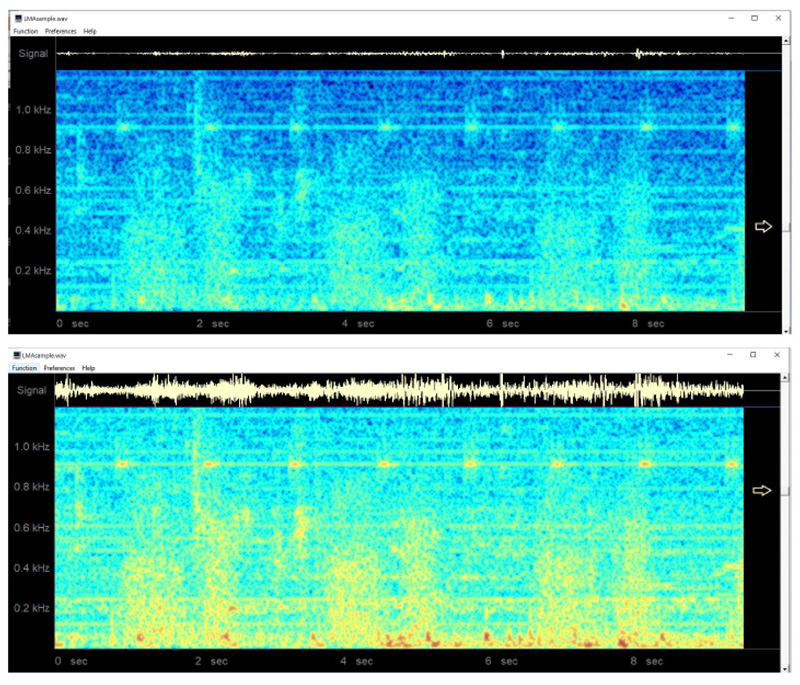
Clinicians who would like to explore the possibilities offered by this nascent technology will likely find that the method is remarkably straight-forward, with only a minimal knowledge of audio recording methods being necessary. That being said, obtaining high-quality color spectrograms for respiratory acoustic data requires attention to a number of technical details. These include the various parameters that are shown in Fig. (11), taken from the spectrogram16 program, as well as appropriately adjusting the system sensitivity ( Fig. 12).
It should also be noted that there are several iPhone and Android apps that produce color spectrograms, in case one wants to avoid using a Windows computer as a computing platform.
Finally, note that the results obtained here, while clearly preliminary in nature by virtue of being a proof-of-concept undertaking, suggest a variety of avenues for possible future studies. These studies might include developing methods to show how color spectrograms might best be processed to reliably detect apnea, snoring or otherwise disordered breathing, to measure the respiratory rate or estimate breath-by-breath tidal volumes. Computer algorithms aimed at filling these needs would likely need to account for interindividual differences in breath sounds and the presence of background noise in the signals.
CONCLUSION
The proof-of-concept spectrographic displays of respiratory sounds, obtained from various sites, suggest that acoustical color spectrographic analysis is a promising future technology for respiratory monitoring.
NOTES
CONSENT FOR PUBLICATION
Not applicable.
AVAILABILITY OF DATA AND MATERIALS
Not applicable.
FUNDING
Funding for this project was entirely from departmental and personal sources.
CONFLICTS OF INTEREST
The author declares that he has no conflicts of interest, financial or otherwise.
ACKNOWLEDGEMENTS
This article is a greatly expanded version of a newsletter contribution available online at: https:// society-for-airway-management.dcatalog.com/ v/ SUMMER-2018-AIRWAY-GAZETTE.